This description is really long?
Team: Brian Bonilla, Byron Flynn, Matt krecicki
Project Motivation
The current power reactor fleet in the United States is rapidly aging. By 2035 our nuclear power generation capacity is projected to be cut in half. This is a major problem due to the fact that nuclear energy accounts for sixty percent of the carbon free energy produced in the United States. In order to maintain our base load power generation ability and the majority of our carbon free energy new reactors must be constructed. Light water reactors have many inherent safety problems such as loss of coolant accidents, and loss of pressurization. In order to avoid possible future Three Mile Island and Fukushima events, non-light water reactors should be constructed when rebuilding the nuclear infrastructure. The new reactor fleet should utilize passive safety features to ensure that a radioactive release is impossible, while still having comparable performance characteristics to light water reactors.
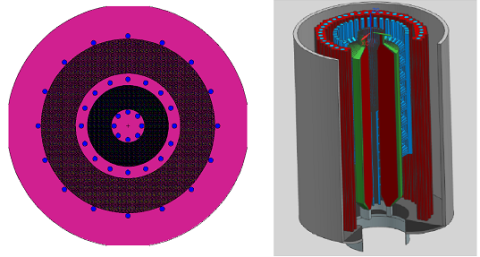
Left Hand Side: MCNP Core Configuration, Right Hand Side: CAD 3D Reactor Design.
Project Description
The proposed solution is a molten salt cooled hybrid pebble-bed and prismatic core reactor which can competitively compete against the Gen III+ reactor designs in the nuclear market. For this to occur the hybrid reactor must be able to have a higher thermal to electric conversion efficiency, decrease capital costs associated with construction, and have a lower probability of a system failure occurrence. The focus of our project is to create a theoretically functioning and safe to run reactor design. The current steam powered turbine reactors have an efficiency of approximately 33% and the proposed hybrid reactor is expected to have an efficiency of about 40%. This is due to the fact that the hybrid reactor can run on a CO2 brayton cycle. Figure 1 shows the proposed reactor design. The left side of Figure 1 shows and over head MCNP view of the core with the purple sections representing the graphite reflectors, the blue sections representing control rod placements and the darker shaded regions are the fuel regions in the core.On the right side there is a NX 3D model of the reactor. The crimson red sections in the model are homogenized Triso fuel regions. The light blue colored parts in the model are the coolant flow paths. The green shaded area is the graphite pebble bed inside the pebble bed section of the reactor. The gray area surrounding the reactor is the 316 stainless steel shell of the reactor. The void area is where the graphite reflectors would be, but are hidden in the picture for visual purposes.The pebble bed section of the reactor has been cut into slices in order to simulate a porous flow since no porous flow model has yet to be generated. The percent of coolant to fuel in the model was based off of the Mark 1 Pebble-Bed and St. Vrain reactors. Flow in both the Mark 1 Pebble-Bed and St. Vrain reactors will be moving upwards along with the Triso pebbles and graphite pebbles. Passive safety features for this design include natural convection, molten salt coolant, graphite core, refractory coated (TRISO) particle fuel, and negative temperature coefficient of reactivity. The natural convection to reduce temperature is due to the DRACS (Direct Reactor Auxiliary Cooling System) heat exchanger, which allows decay heat removal by the DRACS loop. The usage of molten salt coolant allows for a low pressure system to be used as opposed to a high pressure gas cooled reactor decreasing potential risk of damaging a system due to overpressurization. The graphite core provides a high heat capacity, slow thermal response, is a good absorber of tritium, and is able to maintain structural stability at very high temperatures. Another passive safety system includes the use of Triso fuel which allows for extremely high burnup and fission product retention in the pellets even at temperatures higher than that of normal operation. The Triso fuel also is designed to take into account the effects of doppler broadening. As the fuel heats up doppler broadening makes it more likely that fast or epithermal neutrons are absorbed. Because of this, there are less neutrons to start fission reactions which in term lowers the power of the reactor. In the case of an accident scenario the reactor will inherently shut itself down to a safe power-level when the core is above normal operating temperatures. In short, the core has a negative temperature coefficient of reactivity.
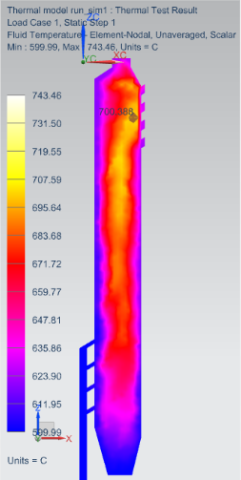
Steady State Thermal Profile oF FLiBe While Flowing Through a Porous Triso Region
Results and Accomplishments
Through the use of Siemens NX’s advanced simulation feature, the thermal hydraulic models of both the pebble bed and prismatic regions were solved. After running the simulations with the theoretically calculated values for mass flow rate, power output per particle, geometric constraints, etc., the resulting thermal core map shown in Figure 2, showed a reasonable thermal profile which fall inside our design constraints/limitations. The change in temperature though the core was found to be approximately 100 degrees celsius. MCNP6 was used to model to the neutronics of the reactor. A full core model was used during simulations. A Matlab script was developed to automate reactor geometry, fuel composition, calculation card, material density, and cell temperature changes. The passive reactivity safety system was proven to have a negative reactivity worth of 1000 pcm.